- 1Infection and Immunity Program, Monash Biomedicine Discovery Institute, Monash University, Clayton, VIC, Australia
- 2Department of Microbiology, Monash University, Clayton, VIC, Australia
- 3Department of Biochemistry and Molecular Biology, Monash University, Clayton, VIC, Australia
In the bacterial flagellar motor, the cell-wall-anchored stator uses an electrochemical gradient across the cytoplasmic membrane to generate a turning force that is applied to the rotor connected to the flagellar filament. Existing theoretical concepts for the stator function are based on the assumption that it anchors around the rotor perimeter by binding to peptidoglycan (P). The existence of another anchoring region on the motor itself has been speculated upon, but is yet to be supported by binding studies. Due to the recent advances in electron cryotomography, evidence has emerged that polar flagellar motors contain substantial proteinaceous periplasmic structures next to the stator, without which the stator does not assemble and the motor does not function. These structures have a morphology of disks, as is the case with Vibrio spp., or a round cage, as is the case with Helicobacter pylori. It is now recognized that such additional periplasmic components are a common feature of polar flagellar motors, which sustain higher torque and greater swimming speeds compared to peritrichous bacteria such as Escherichia coli and Salmonella enterica. This review summarizes the data available on the structure, composition, and role of the periplasmic scaffold in polar bacterial flagellar motors and discusses the new paradigm for how such motors assemble and function.
Overview of the Bacterial Flagellum
The flagellum (Figure 1) comprises the basal body, hook, and filament. The basal body functions as a rotary motor; the turning force (torque) generated by it is transmitted through the hook to the filament, causing it to spin (Zhao et al., 2014; Carroll and Liu, 2020; Takekawa et al., 2020). Four main types of flagellar arrangement have been observed: monotrichious bacteria (e.g., Vibrio cholerae) carry a single polar flagellum; amphitrichous cells (Campylobacter jejuni) have one or more flagella at both poles; lophotrichous bacteria (Helicobacter pylori) have multiple flagella at one pole; while peritrichous bacteria (Escherichia coli) possess multiple flagella distributed over the cell envelope (Schuhmacher et al., 2015).
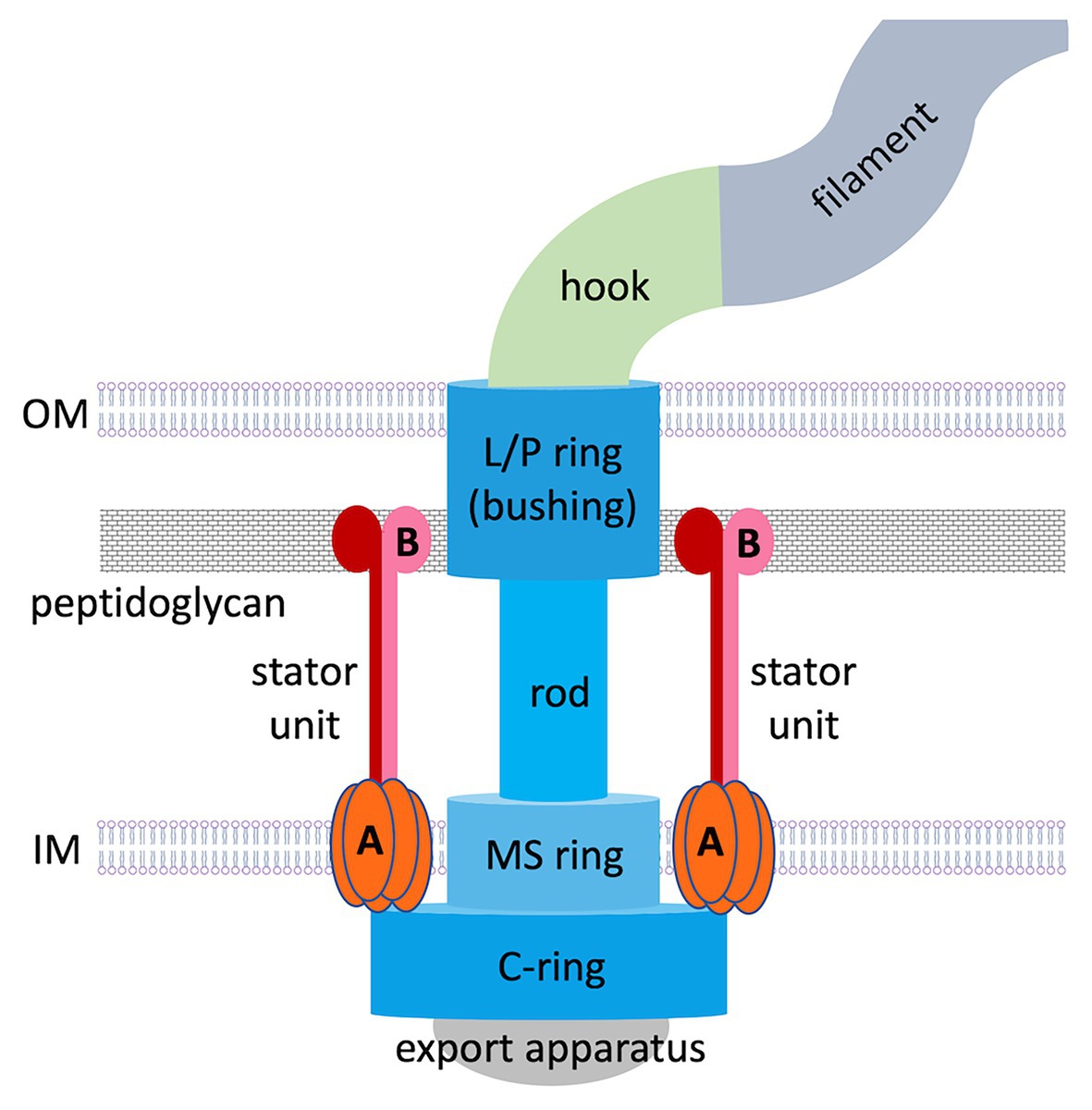
Figure 1. Overall structure of a prototypical flagellar motor in Gram-negative bacteria. Basal body components are colored in shades of blue. Stator components (A, MotA; B, MotB) are colored in shades of red.
The flagellar motor is a remarkable nanoscale molecular engine that self-assembles in the cell wall from many protein components. Current knowledge about its structure and function has been largely acquired from studies on peritrichous bacteria. Flagellar assembly begins with the membrane/supramembrane (MS) ring, export apparatus and switch complex [also known as the cytoplasmic ring (C-ring); Kubori et al., 1992; Li and Sourjik, 2011]. Then the rod and hook are assembled by transporting their components via the export apparatus (Minamino et al., 2008), a bushing for the rod [comprising peptidoglycan (P) and outer membrane (OM; lipopolysaccharide, L) rings] is added, and stator units assemble in a ring around the rotor perimeter.
The stator ring generates torque using an electrochemical gradient of protons or sodium ions across the inner membrane (IM). In proton-motive-force-driven motors, a single stator unit is an asymmetric assembly of five MotA subunits enclosing two MotB subunits (Deme et al., 2020; Santiveri et al., 2020). In sodium-motive-force-driven motors, the stator is composed of PomAB or MotPS complexes of the same stoichiometry. Existing theoretical concepts for why the stator itself does not spin are based on the assumption that it is anchored to the cell wall by MotB/PomB/MotS binding to P (Roujeinikova, 2008; O’Neill et al., 2011; Reboul et al., 2011; Andrews et al., 2017; Deme et al., 2020; Santiveri et al., 2020). However, visualization of the intact flagellar motor in whole cells (in situ), made possible by recent advances in electron cryotomography, revealed that polar flagellar motors contain a substantial proteinaceous periplasmic structure next to the stator (Murphy et al., 2006; Liu et al., 2009; Chen et al., 2011; Beeby et al., 2016; Qin et al., 2017), which has a morphology of disks, rings, or a round cage. It is now recognized that additional periplasmic components are a common feature of polar motors, which sustain higher torque and greater swimming speeds compared to peritrichous bacteria. Evidence has emerged that this periplasmic scaffold may serve as another anchoring region for the stator units. This review summarizes the data available of the structure, composition, and role of the periplasmic scaffold in polar bacterial flagellar motors and discusses the new paradigm for how these motors assemble and function.
Early Studies: The Discovery of Additional Periplasmic Disks
First evidence that polar flagellar motors possess additional periplasmic components that may be required for their function emerged from electron microscopy (EM) studies of bacterial preparations. Coulton and Murray (1977) observed proteinaceous concentric rings positioned laterally to the basal bodies in (Aqua)spirillum serpens. These rings formed 90-nm diameter disks associated with the periplasmic face of the OM. Subsequently, double-layered 90–150-nm diameter disks were observed at the same position in the motors of C. jejuni and Campylobacter (Vibrio) fetus subsp. intestinalis (Morooka et al., 1983). A similar disk was discovered in the motors of V. cholerae (Ferris et al., 1984) and Wolinella succinogenes (Engelhardt et al., 1993), but while the diameter of the former did not exceed 41 nm, the average diameter of the latter was 170 nm, suggesting the architecture of this structure is species-specific.
Work on W. succinogenes was important, because it showed that the additional disks (from then on referred to as basal disks) are attached not only to the periplasmic face of the OM, but also to the basal body, and that the L/P disk (bushing) is integrated at the center of the basal disk (Kupper et al., 1989; Engelhardt et al., 1993). By that point, studies converged on the hypothesis that the basal disk may serve to anchor the L/P bushing of the motor to the cell wall and ensure correct positioning of the stator units around the rotor.
Interestingly, early work identified basal disks only in proteobacteria with polar flagella, but the possibility that additional periplasmic structures may exist in other flagellated bacteria could not be discounted. The discovery and characterization of such components was hampered by the fact that many are lost in the process of isolation of flagella for analysis by negative staining. It was not until recently that new techniques, such as electron cryotomography and high-throughput genome sequencing, could provide a detailed picture of the entire flagellar motor.
First Electron Cryotomography Motor Reconstructions: The Discovery and Classification of Periplasmic Scaffolds
The electron cryotomography technique (Oikonomou and Jensen, 2017) has a distinct advantage over traditional transmission EM methods as it allows visualization of the entire flagellar motor in frozen whole cells, without the need for fixation, dehydration, or staining. In 2006, a pioneering study of the spirochaete Treponema primitia provided the first 3D reconstruction of the polar motor that included the stator (Murphy et al., 2006). It also revealed a novel periplasmic structure next to the stator, termed the collar, which appears to be unique to Spirochaetes. Furthermore, the observed size of the stator on its periplasmic side could not be accounted for by MotB only, suggesting the presence of some other proteins. It was hypothesized that these extra structures serve as a periplasmic scaffold that recruits, organizes, and stabilizes the stator units. A subsequent electron cryotomography survey of the motor architectures (Chen et al., 2011) and related studies by other labs (Liu et al., 2009, 2010; Raddi et al., 2012) revealed that periplasmic scaffolds exist in the polar motors of many other species, but are absent in the motors of peritrichous bacteria, and that many polar flagellar motors are significantly more complex than the prototypical motors of E. coli and Salmonella enterica.
Flagellar motors can be classified according to four scaffold types: non-scaffold motors (Figures 2A,B); OM-associated scaffold motors (Figures 2C,D); IM-associated scaffold motors (Figures 2E,F); and integrated scaffold motors (Figures 2G,H).
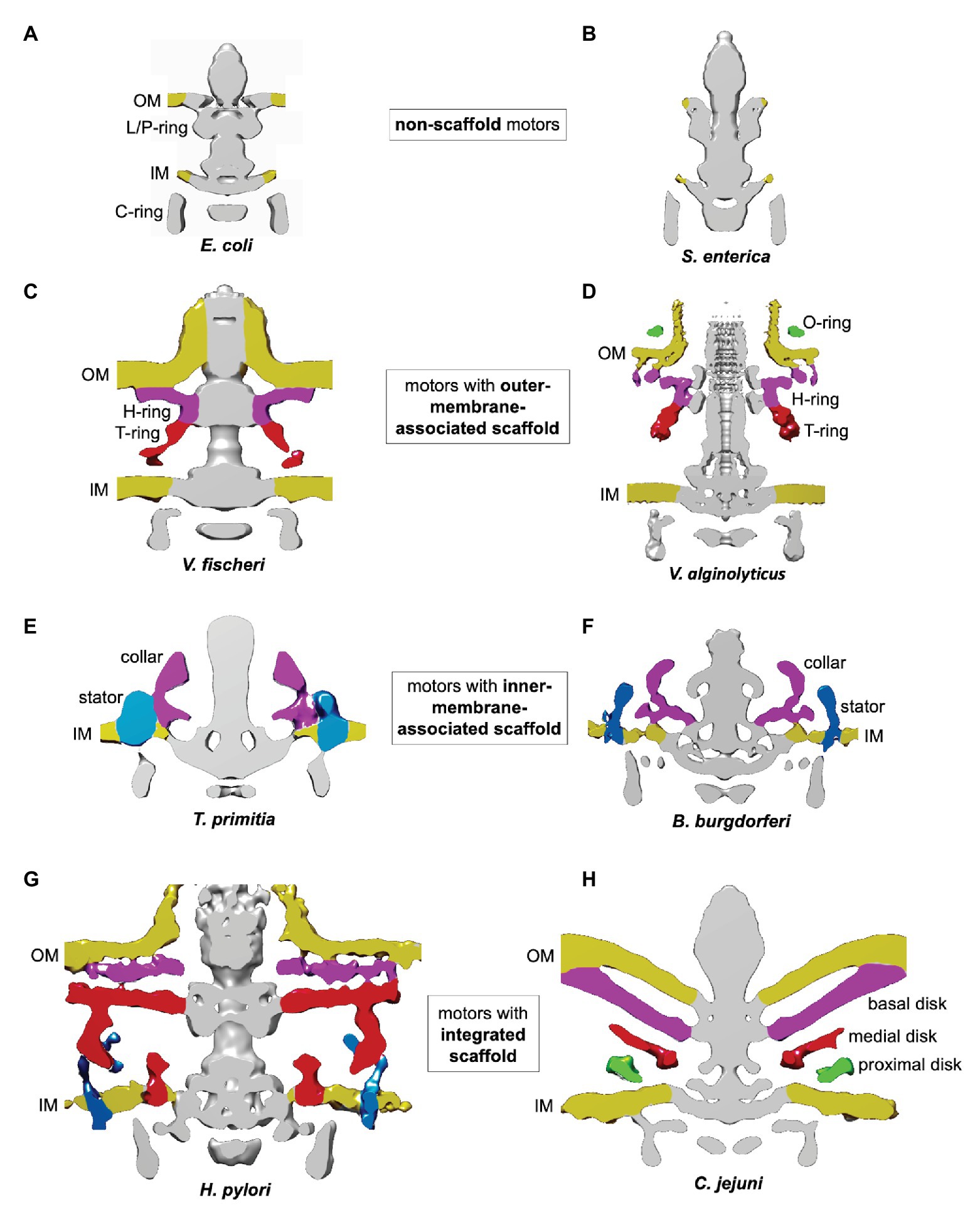
Figure 2. Classification of flagellar motors based on their scaffolds. The figures were prepared using electron cryotomography maps for the flagellar motors from: (A) Escherichia coli (EMDB-5311; Chen et al., 2011); (B) Salmonella enterica (EMDB-5310; Chen et al., 2011); (C) Vibrio fischeri (EMDB 3155; Beeby et al., 2016); (D) Vibrio alginolyticus (EMDB-21027 and EMDB-21819; Carroll et al., 2020; Zhu et al., 2020); (E) Treponema primitia (EMDB-1235; Murphy et al., 2006); (F) Borrelia burgdorferi (EMDB-9122; Qin et al., 2018); (G) Helicobacter pylori (EMDB-8459; Qin et al., 2017); and (H) Campylobacter jejuni (EMDB-3150; Beeby et al., 2016).
An example of an OM-associated scaffold is the H/T/O-ring system found in the polar motor in Vibrio alginolyticus (Zhu et al., 2017, 2020; Figure 2D). The O-ring, associated with the external face of the OM, surrounds the base of the hook. The periplasmic H-ring, associated with the inner face of the OM, surrounds the L/P-ring. The periplasmic T-ring assembles at the outer rim of the H-ring, below the P-ring.
Inner-membrane-associated scaffolds have been found in polar motors driving the periplasmic flagella in Spirochaetes (Murphy et al., 2006; Liu et al., 2009, 2010; Raddi et al., 2012). They are exemplified by the collar-like structures seen in T. primitia (Figure 2E) and Borrelia burgdorferi (Qin et al., 2018; Figure 2F). The narrow part of these structures is embedded into the IM between the MS and stator rings, while the wider rim is positioned in the periplasm.
An integrated scaffold, spanning the periplasm and associated with both OM and IM, has been found in the polar motors of the closely related bacteria H. pylori and C. jejuni (Beeby et al., 2016; Qin et al., 2017; Figures 2G,H). The core of the scaffold is a round cage-like structure encircling the rod, the MS, and L/P rings. It is anchored to the IM on one side, and extends to the basal disk associated with the periplasmic face of the OM on the other.
Molecular Composition of Periplasmic Scaffolds
The OM-associated scaffold of the Vibrio spp. motor has been dissected in detail. The T-ring comprises MotX and MotY (Terashima et al., 2006), while the adjacent H-ring is made up of the OM lipoproteins (OMLPs) FlgO and FlgP and the periplasmic protein FlgT (Terashima et al., 2013; Beeby et al., 2016; Zhu et al., 2018, 2020). The protein make-up of the O-ring is not yet known.
The IM-associated scaffold of the spirochaetal motor has been studied extensively in B. burgdorferi. The base of the collar contains FlbB (Chen et al., 2011; Moon et al., 2016), which is anchored to the IM via its N-terminal transmembrane helix. Although the full protein composition of the collar is not yet known, the tetratricopeptide repeat (TPR) proteins BB0236 (Moon et al., 2018) and FlcA (Xu et al., 2020) have been identified as putative collar proteins that assemble onto the FlbB base.
The molecular composition of the integrated scaffold of the H. pylori motor is yet to be established. However, it is in a similar position to the integrated scaffold seen in C. jejuni (Figures 2G,H), and it is likely that the two scaffolds share at least some common components. The three main parts of the C. jejuni scaffold are: the basal disk associated with the periplasmic face of the OM; a medial disk around the rod; and a disk proximal to the IM (Figure 2H). The basal disk is formed by the OMLP FlgP (homologous to Vibrio FlgP), likely in complex with FlgQ (Beeby et al., 2016). The medial disk is composed of paralyzed flagellum protein A (PflA), a periplasmic TPR protein. The IM-proximal disk contains the TPR protein PflB, which is anchored to the IM via a single transmembrane helix. Proteins homologous to C. jejuni FlgP, PflA, and PflB are present in H. pylori (Rajagopala et al., 2007; Sommerlad and Hendrixson, 2007), suggesting C. jejuni and H. pylori scaffolds assemble in a similar manner.
The Role of the Individual Scaffold Components
OM-Associated Scaffold of the Vibrio Motor
ΔflgT mutant cells lacked the H-ring and mostly produced periplasmic, rather than native, external flagella (Terashima et al., 2010; Zhu et al., 2018). Most of the H-ring was missing in ΔflgP mutant cells, which also lacked external hook/filament structures (Beeby et al., 2016). Thus, one apparent function of the H-ring is to mediate the OM penetration during the flagellum biogenesis. This notion is strengthened by reports that mutations in flgO also resulted in a reduced number of cells with external flagella (Martinez et al., 2009; Zhu et al., 2018). The other putative function of the H-ring is to anchor the flagellum to the cell wall, by associating with the OM via its medial part containing the OMLP FlgP (Morris et al., 2008), and its outer part, containing the OMLP FlgO (Martinez et al., 2009; Zhu et al., 2018). In addition, the H-ring is required for stator ring assembly (Beeby et al., 2016).
The inner part of the T-ring is formed by MotY (Terashima et al., 2006; Zhu et al., 2017), which is likely anchored to peptidoglycan, as it contains a peptidoglycan-binding motif (Okunishi et al., 1996; Kojima et al., 2008). The stator-proximal edge of the T-ring is formed by MotX (Terashima et al., 2006; Zhu et al., 2017). In the absence of MotX or MotY, bacteria were non-motile (Gosink and Häse, 2000), and the stator units were not recruited to the cell pole (Terashima et al., 2006), indicating that the T-ring is also required for stator assembly.
Inner-Membrane-Associated Scaffold of the Spirochaetal Motor
ΔflbB, Δbb0236, and ΔflcA mutant cells had less flagella per cell than the wild type, suggesting that all three putative collar components play an important role in the flagella biogenesis (Moon et al., 2016, 2018; Xu et al., 2020). Furthermore, all three mutants were non-motile, lacked the stator, and produced flagella that were abnormally oriented toward the cell pole rather than to the cell cylinder. This shows that the collar is required for correct orientation of the periplasmic flagella and assembly of the stator in B. burgdorferi.
Integrated Scaffold of the Campylobacterotal Motor
The basal disk in the integrated motor scaffold in C. jejuni is thought to play a similar role to that of the H-disk in the OM-associated scaffold in the Vibrio motor. Both structures contain the OMLP FlgP, although the C. jejuni and Vibrio proteins share only limited sequence similarity (Beeby et al., 2016). Deletion of flgP resulted in the loss of the disk and loss of functionality in both types of the motor, but in contrast to the Vibrio fischeri ΔflgP mutant, which lacked external hook/filament structures, the ΔflgP mutant of C. jejuni assembled normal-looking flagella (Sommerlad and Hendrixson, 2007). Another difference is that in the absence of the H-ring, the remainder of the scaffold (the T-ring) still assembled at least in some Vibrio species (Zhu et al., 2018), whereas the loss of the basal ring in C. jejuni resulted in the loss of the entire scaffold (Beeby et al., 2016). Despite the differences, the loss of function in ΔflgP mutants with either type of motor is attributable to the fact that the stator does not assemble in the absence of the basal ring or H-ring (Martinez et al., 2010).
The medial disk containing PflA is required for assembly of the proximal disk containing PflB, and ΔpflA mutants are non-motile (Yao et al., 1994) because their motors lack the stators (Beeby et al., 2016). MotB was shown to incorporate into the motor only in the presence of the proximal ring, supporting the hypothesis that upon assembly into the polar motor of C. jejuni, the stator units are anchored to the integrated scaffold via PflB.
Implications For the Mechanism of Generation of Higher Torque in Polar Flagellar Motors
Thus, due to recent advances in electron cryotomography, extensive evidence has emerged that in contrast to peritrichous flagella, polar flagellar motors evolved diverse periplasmic scaffolds around the rotor, without which the stator does not assemble and the motor does not function. It is now widely accepted that upon assembly into the polar motors the stator units are anchored not only to peptidoglycan, but also to these scaffolds, and despite unique differences between the architectures of the OM-associated, IM-associated and integrated scaffolds, commonalities start to emerge with regards to their role in the stator function.
It is now recognized that the number of stator units recruited into the motor determines the total torque: the higher the number, the higher the force (Lele et al., 2013). Furthermore, evidence has emerged that, owing to the presence of the stabilizing scaffolds, stator rings in many polar motors are wider than in peritrichous motors (Chen et al., 2011; Beeby et al., 2016; Qin et al., 2017; Chaban et al., 2018; Chang et al., 2019). We now understand that larger-diameter stator rings not only accommodate more stator complexes, but also place them further away from the axis, resulting in a higher momentum of force produced by each complex, and hence higher overall torque. In peritrichous flagella of S. enterica and E. coli, at least 11 stator complexes can anchor to the peptidoglycan layer and P-ring (Reid et al., 2006; Hizukuri et al., 2010; O’Neill et al., 2011) and apply force on the 40-nm-diameter C-ring, producing ~1,300 pN nm torque (Reid et al., 2006; Thomas et al., 2006). In comparison, the C-ring in the polar motor of H. pylori, for example, is significantly larger (57 nM) and surrounded by half as many stator complexes (18; Qin et al., 2017), which is consistent with the observed higher torque (~3,600 pN nm; Celli et al., 2009). The C-ring in the spirochaetal polar motor is similarly large and surrounded by 16 stator complexes (Zhao et al., 2014; Chang et al., 2019), producing a torque of ~4,000 pN nm (Nakamura et al., 2014). Thus, the accumulated structural and functional data on the periplasmic scaffolds in the polar motors are consistent with their role as platforms that recruit a wider power ring to sustain higher torque.
Implications For Evolutionary Adaptation
The remarkable structural diversity of scaffolds in polar motors suggests they have evolved from a less complex ancestral motor, composed of the common core components seen today in peritrichous motors, by acquiring accessory proteins (Beeby et al., 2020). The existence of class‐ and genera-specific scaffold components (such as MotX/MotY in Vibrio, FlbB in Spirochaetes and PflA/PflB in Campylobacterota) is indicative of distinct evolutionary pathways resulting in motors with mechanical outputs that, when combined with other factors, suit specific habitats. At one end of the torque spectrum is the polar motor of Caulobacter crescentus. To survive in its low-nutrient freshwater habitat, C. crescentus has evolved an efficient motor with no scaffolding structures (Rossmann et al., 2020) that uses a small stator ring comprising only 11 units. The economically low torque (∼350 pN nm; Li and Tang, 2006) is sufficient to propel the cell due to additional thrust created by the helical motion of the cell (Liu et al., 2014). At the other end of the spectrum is the high-torque motor of H. pylori. This microorganism resides within the very viscous mucous layer of the stomach (Hooi et al., 2017) and, apparently through natural selection, demonstrates unusually high motility in viscous media (Hazell et al., 1986). Together with the helical cell shape, the high torque, afforded by the wider stator ring supported by a periplasmic scaffold, allows H. pylori locomotion in high-viscosity environment.
The mechanism by which the scaffolds recruit and stabilize stator complexes remains enigmatic. TPR domains often serve as protein-protein interaction scaffolds (Blatch and Lässle, 1999), and their presence in FlbB, PflA, and PflB is consistent with their proposed stator scaffolding role. Dissecting the 3D architecture of the periplasmic scaffolds and unraveling the structural basis for their ability to recruit stator units will be a fascinating and worthy task. Achieving this goal will advance our knowledge about the mechanism of the bacterial flagellar motor, and our understanding of the convergent evolutionary pathways to higher-torque polar flagellar motors.
Author Contributions
All authors listed have made a substantial, direct and intellectual contribution to the work, and approved it for publication.
Conflict of Interest
The authors declare that the research was conducted in the absence of any commercial or financial relationships that could be construed as a potential conflict of interest.
References
Andrews, D. A., Nesmelov, Y. E., Wilce, M. C., and Roujeinikova, A. (2017). Structural analysis of variant of Helicobacter pylori MotB in its activated form, engineered as chimera of MotB and leucine zipper. Sci. Rep. 7:13435. doi: 10.1038/s41598-017-13421-0
Beeby, M., Ferreira, J. L., Tripp, P., Albers, S. -V., and Mitchell, D. R. (2020). Propulsive nanomachines: the convergent evolution of archaella, flagella and cilia. FEMS Microbiol. Rev. 44, 253–304. doi: 10.1093/femsre/fuaa006
Beeby, M., Ribardo, D. A., Brennan, C. A., Ruby, E. G., Jensen, G. J., and Hendrixson, D. R. (2016). Diverse high-torque bacterial flagellar motors assemble wider stator rings using a conserved protein scaffold. Proc. Natl. Acad. Sci. U. S. A. 113, E1917–E1926. doi: 10.1073/pnas.1518952113
Blatch, G. L., and Lässle, M. (1999). The tetratricopeptide repeat: a structural motif mediating protein-protein interactions. Bioessays 21, 932–939. doi: 10.1002/(SICI)1521-1878(199911)21:11<932::AID-BIES5>3.0.CO;2-N
Carroll, B. L., and Liu, J. (2020). Structural conservation and adaptation of the bacterial flagella motor. Biomolecules 10:1492. doi: 10.3390/biom10111492
Carroll, B. L., Nishikino, T., Guo, W., Zhu, S., Kojima, S., Homma, M., et al. (2020). The flagellar motor of vibrio alginolyticus undergoes major structural remodeling during rotational switching. Elife 9:e61446. doi: 10.7554/eLife.61446
Celli, J. P., Turner, B. S., Afdhal, N. H., Keates, S., Ghiran, I., Kelly, C. P., et al. (2009). Helicobacter pylori moves through mucus by reducing mucin viscoelasticity. Proc. Natl. Acad. Sci. U. S. A. 106, 14321–14326. doi: 10.1073/pnas.0903438106
Chaban, B., Coleman, I., and Beeby, M. (2018). Evolution of higher torque in Campylobacter-type bacterial flagellar motors. Sci. Rep. 8:97. doi: 10.1038/s41598-017-18115-1
Chang, Y., Moon, K. H., Zhao, X., Norris, S. J., Motaleb, M. A., and Liu, J. (2019). Structural insights into flagellar stator-rotor interactions. Elife 8:e48979. doi: 10.7554/eLife.48979
Chen, S., Beeby, M., Murphy, G. E., Leadbetter, J. R., Hendrixson, D. R., Briegel, A., et al. (2011). Structural diversity of bacterial flagellar motors. EMBO J. 30, 2972–2981. doi: 10.1038/emboj.2011.186
Coulton, J. W., and Murray, R. G. (1977). Membrane-associated components of the bacterial flagellar apparatus. Biochim. Biophys. Acta 465, 290–310. doi: 10.1016/0005-2736(77)90080-3
Deme, J. C., Johnson, S., Vickery, O., Aron, A., Monkhouse, H., Griffiths, T., et al. (2020). Structures of the stator complex that drives rotation of the bacterial flagellum. Nat. Microbiol. 5, 1553–1564. doi: 10.1038/s41564-020-0788-8
Engelhardt, H., Schuster, S. C., and Baeuerlein, E. (1993). An archimedian spiral: the basal disk of the wolinella flagellar motor. Science 262, 1046–1048. doi: 10.1126/science.8235620
Ferris, F. G., Beveridge, T. J., Marceau-Day, M. L., and Larson, A. D. (1984). Structure and cell envelope associations of flagellar basal complexes of Vibrio cholerae and Campylobacter fetus. Can. J. Microbiol. 30, 322–333. doi: 10.1139/m84-048
Gosink, K. K., and Häse, C. C. (2000). Requirements for conversion of the Na+-driven flagellar motor of Vibrio cholerae to the H+-driven motor of Escherichia coli. J. Bacteriol. 182, 4234–4240. doi: 10.1128/JB.182.15.4234-4240.2000
Hazell, S. L., Lee, A., Brady, L., and Hennessy, W. (1986). Campylobacter pyloridis and gastritis: association with intercellular spaces and adaptation to an environment of mucus as important factors in colonization of the gastric epithelium. J. Infect. Dis. 153, 658–663. doi: 10.1093/infdis/153.4.658
Hizukuri, Y., Kojima, S., and Homma, M. (2010). Disulphide cross-linking between the stator and the bearing components in the bacterial flagellar motor. J. Biochem. 148, 309–318. doi: 10.1093/jb/mvq067
Hooi, J. K. Y., Lai, W. Y., Ng, W. K., Suen, M. M. Y., Underwood, F. E., Tanyingoh, D., et al. (2017). Global prevalence of Helicobacter pylori infection: systematic review and meta-analysis. Gastroenterology 153, 420–429. doi: 10.1053/j.gastro.2017.04.022
Kojima, S., Shinohara, A., Terashima, H., Yakushi, T., Sakuma, M., Homma, M., et al. (2008). Insights into the stator assembly of the Vibrio flagellar motor from the crystal structure of MotY. Proc. Natl. Acad. Sci. U. S. A. 105, 7696–7701. doi: 10.1073/pnas.0800308105
Kubori, T., Shimamoto, N., Yamaguchi, S., Namba, K., and Aizawa, S. -I. (1992). Morphological pathway of flagellar assembly in Salmonella typhimurium. J. Mol. Biol. 226, 433–446. doi: 10.1016/0022-2836(92)90958-M
Kupper, J., Wildhaber, I., Gao, Z., and Baeuerlein, E. (1989). Basal-body-associated disks are additional structural elements of the flagellar apparatus isolated from Wolinella succinogenes. J. Bacteriol. 171, 2803–2810. doi: 10.1128/JB.171.5.2803-2810.1989
Lele, P. P., Hosu, B. G., and Berg, H. C. (2013). Dynamics of mechanosensing in the bacterial flagellar motor. Proc. Natl. Acad. Sci. U. S. A. 110, 11839–11844. doi: 10.1073/pnas.1305885110
Li, H., and Sourjik, V. (2011). Assembly and stability of flagellar motor in Escherichia coli. Mol. Microbiol. 80, 886–899. doi: 10.1111/j.1365-2958.2011.07557.x
Li, G., and Tang, J. X. (2006). Low flagellar motor torque and high swimming efficiency of Caulobacter crescentus swarmer cells. Biophys. J. 91, 2726–2734. doi: 10.1529/biophysj.106.080697
Liu, B., Gulino, M., Morse, M., Tang, J. X., Powers, T. R., and Breuer, K. S. (2014). Helical motion of the cell body enhances Caulobacter crescentus motility. Proc. Natl. Acad. Sci. U. S. A. 111, 11252–11256. doi: 10.1073/pnas.1407636111
Liu, J., Howell, J. K., Bradley, S. D., Zheng, Y., Zhou, Z. H., and Norris, S. J. (2010). Cellular architecture of Treponema pallidum: novel flagellum, periplasmic cone, and cell envelope as revealed by cryo electron tomography. J. Mol. Biol. 403, 546–561. doi: 10.1016/j.jmb.2010.09.020
Liu, J., Lin, T., Botkin, D. J., McCrum, E., Winkler, H., and Norris, S. J. (2009). Intact flagellar motor of Borrelia burgdorferi revealed by cryo-electron tomography: evidence for stator ring curvature and rotor/C-ring assembly flexion. J. Bacteriol. 191:5026. doi: 10.1128/JB.00340-09
Martinez, R. M., Dharmasena, M. N., Kirn, T. J., and Taylor, R. K. (2009). Characterization of two outer membrane proteins, FlgO and FlgP, that influence Vibrio cholerae motility. J. Bacteriol. 191, 5669–5679. doi: 10.1128/JB.00632-09
Martinez, R. M., Jude, B. A., Kirn, T. J., Skorupski, K., and Taylor, R. K. (2010). Role of FlgT in anchoring the flagellum of Vibrio cholerae. J. Bacteriol. 192, 2085–2092. doi: 10.1128/JB.01562-09
Minamino, T., Imada, K., and Namba, K. (2008). Mechanisms of type III protein export for bacterial flagellar assembly. Mol. Biosyst. 4, 1105–1115. doi: 10.1039/b808065h
Moon, K. H., Zhao, X., Manne, A., Wang, J., Yu, Z., Liu, J., et al. (2016). Spirochetes flagellar collar protein FlbB has astounding effects in orientation of periplasmic flagella, bacterial shape, motility, and assembly of motors in Borrelia burgdorferi. Mol. Microbiol. 102, 336–348. doi: 10.1111/mmi.13463
Moon, K. H., Zhao, X., Xu, H., Liu, J., and Motaleb, M. A. (2018). A tetratricopeptide repeat domain protein has profound effects on assembly of periplasmic flagella, morphology and motility of the Lyme disease spirochete Borrelia burgdorferi. Mol. Microbiol. 110, 634–647. doi: 10.1111/mmi.14121
Morooka, T., Umeda, A., and Amako, K. (1983). Morphological differences in flagella in Campylobacter fetus subsp. intestinalis and C. fetus subsp. jejuni. Microbiol. Immunol. 27, 655–662. doi: 10.1111/j.1348-0421.1983.tb00628.x
Morris, D. C., Peng, F., Barker, J. R., and Klose, K. E. (2008). Lipidation of an FlrC-dependent protein is required for enhanced intestinal colonization by Vibrio cholerae. J. Bacteriol. 190, 231–239. doi: 10.1128/JB.00924-07
Murphy, G. E., Leadbetter, J. R., and Jensen, G. J. (2006). In situ structure of the complete Treponema primitia flagellar motor. Nature 442, 1062–1064. doi: 10.1038/nature05015
Nakamura, S., Leshansky, A., Magariyama, Y., Namba, K., and Kudo, S. (2014). Direct measurement of helical cell motion of the spirochete Leptospira. Biophys. J. 106, 47–54. doi: 10.1016/j.bpj.2013.11.1118
Oikonomou, C. M., and Jensen, G. J. (2017). Cellular electron cryotomography: toward structural biology in situ. Annu. Rev. Biochem. 86, 873–896. doi: 10.1146/annurev-biochem-061516-044741
Okunishi, I., Kawagishi, I., and Homma, M. (1996). Cloning and characterization of motY, a gene coding for a component of the sodium-driven flagellar motor in Vibrio alginolyticus. J. Bacteriol. 178, 2409–2415. doi: 10.1128/JB.178.8.2409-2415.1996
O’Neill, J., Xie, M., Hijnen, M., and Roujeinikova, A. (2011). Role of the MotB linker in the assembly and activation of the bacterial flagellar motor. Acta Crystallogr. D Biol. Crystallogr. 67, 1009–1016. doi: 10.1107/S0907444911041102
Qin, Z., Lin, W. -t., Zhu, S., Franco, A. T., Liu, J., and Zhulin, I. B. (2017). Imaging the motility and chemotaxis machineries in Helicobacter pylori by cryo-electron tomography. J. Bacteriol. 199:e00695–16. doi: 10.1128/JB.00695-16
Qin, Z., Tu, J., Lin, T., Norris, S. J., Li, C., Motaleb, M. A., et al. (2018). Cryo-electron tomography of periplasmic flagella in Borrelia burgdorferi reveals a distinct cytoplasmic ATPase complex. PLoS Biol. 16:e3000050. doi: 10.1371/journal.pbio.3000050
Raddi, G., Morado, D. R., Yan, J., Haake, D. A., Yang, X. F., and Liu, J. (2012). Three-dimensional structures of pathogenic and saprophytic Leptospira species revealed by cryo-electron tomography. J. Bacteriol. 194, 1299–1306. doi: 10.1128/jb.06474-11
Rajagopala, S. V., Titz, B., Goll, J., Parrish, J. R., Wohlbold, K., McKevitt, M. T., et al. (2007). The protein network of bacterial motility. Mol. Syst. Biol. 3:128. doi: 10.1038/msb4100166
Reboul, C. F., Andrews, D. A., Nahar, M. F., Buckle, A. M., and Roujeinikova, A. (2011). Crystallographic and molecular dynamics analysis of loop motions unmasking the peptidoglycan-binding site in stator protein MotB of flagellar motor. PLoS One 6:e18981. doi: 10.1371/journal.pone.0018981
Reid, S. W., Leake, M. C., Chandler, J. H., Lo, C. J., Armitage, J. P., and Berry, R. M. (2006). The maximum number of torque-generating units in the flagellar motor of Escherichia coli is at least 11. Proc. Natl. Acad. Sci. U. S. A. 103, 8066–8071. doi: 10.1073/pnas.0509932103
Rossmann, F. M., Hug, I., Sangermani, M., Jenal, U., and Beeby, M. (2020). In situ structure of the Caulobacter crescentus flagellar motor and visualization of binding of a CheY-homolog. Mol. Microbiol. 114, 443–453. doi: 10.1111/mmi.14525
Roujeinikova, A. (2008). Crystal structure of the cell wall anchor domain of MotB, a stator component of the bacterial flagellar motor: implications for peptidoglycan recognition. Proc. Natl. Acad. Sci. U. S. A. 105, 10348–10353. doi: 10.1073/pnas.0803039105
Santiveri, M., Roa-Eguiara, A., Kühne, C., Wadhwa, N., Hu, H., Berg, H. C., et al. (2020). Structure and function of stator units of the bacterial flagellar motor. Cell 183, 244.e16–257.e16. doi: 10.1016/j.cell.2020.08.016
Schuhmacher, J. S., Thormann, K. M., and Bange, G. (2015). How bacteria maintain location and number of flagella? FEMS Microbiol. Rev. 39, 812–822. doi: 10.1093/femsre/fuv034
Sommerlad, S. M., and Hendrixson, D. R. (2007). Analysis of the roles of FlgP and FlgQ in flagellar motility of Campylobacter jejuni. J. Bacteriol. 189, 179–186. doi: 10.1128/JB.01199-06
Takekawa, N., Imada, K., and Homma, M. (2020). Structure and energy-conversion mechanism of the bacterial Na+-driven flagellar motor. Trends Microbiol. 28, 719–731. doi: 10.1016/j.tim.2020.03.010
Terashima, H., Fukuoka, H., Yakushi, T., Kojima, S., and Homma, M. (2006). The Vibrio motor proteins, MotX and MotY, are associated with the basal body of Na+-driven flagella and required for stator formation. Mol. Microbiol. 62, 1170–1180. doi: 10.1111/j.1365-2958.2006.05435.x
Terashima, H., Koike, M., Kojima, S., and Homma, M. (2010). The flagellar basal body-associated protein FlgT is essential for a novel ring structure in the sodium-driven Vibrio motor. J. Bacteriol. 192, 5609–5615. doi: 10.1128/JB.00720-10
Terashima, H., Li, N., Sakuma, M., Koike, M., Kojima, S., Homma, M., et al. (2013). Insight into the assembly mechanism in the supramolecular rings of the sodium-driven Vibrio flagellar motor from the structure of FlgT. Proc. Natl. Acad. Sci. U. S. A. 110, 6133–6138. doi: 10.1073/pnas.1222655110
Thomas, D. R., Francis, N. R., Xu, C., and DeRosier, D. J. (2006). The three-dimensional structure of the flagellar rotor from a clockwise-locked mutant of Salmonella enterica serovar Typhimurium. J. Bacteriol. 188, 7039–7048. doi: 10.1128/JB.00552-06
Xu, H., He, J., Liu, J., and Motaleb, M. A. (2020). BB0326 is responsible for the formation of periplasmic flagellar collar and assembly of the stator complex in Borrelia burgdorferi. Mol. Microbiol. 113, 418–429. doi: 10.1111/mmi.14428
Yao, R., Burr, D. H., Doig, P., Trust, T. J., Niu, H., and Guerry, P. (1994). Isolation of motile and non-motile insertional mutants of Campylobacter jejuni: the role of motility in adherence and invasion of eukaryotic cells. Mol. Microbiol. 14, 883–893. doi: 10.1111/j.1365-2958.1994.tb01324.x
Zhao, X., Norris, S. J., and Liu, J. (2014). Molecular architecture of the bacterial flagellar motor in cells. Biochemistry 53, 4323–4333. doi: 10.1021/bi500059y
Zhu, S., Nishikino, T., Hu, B., Kojima, S., Homma, M., and Liu, J. (2017). Molecular architecture of the sheathed polar flagellum in Vibrio alginolyticus. Proc. Natl. Acad. Sci. U. S. A. 114, 10966–10971. doi: 10.1073/pnas.1712489114
Zhu, S., Nishikino, T., Kojima, S., Homma, M., and Liu, J. (2018). The Vibrio H-ring facilitates the outer membrane penetration of the polar sheathed flagellum. J. Bacteriol. 200:e00387–18. doi: 10.1128/JB.00387-18
Keywords: bacterial flagellar motor, structure and function, polar flagellum, torque, electron cryotomography
Citation: Zhou X and Roujeinikova A (2021) The Structure, Composition, and Role of Periplasmic Stator Scaffolds in Polar Bacterial Flagellar Motors. Front. Microbiol. 12:639490. doi: 10.3389/fmicb.2021.639490
Edited by:
Matt Arthur Baker, University of New South Wales, AustraliaReviewed by:
Morgan Beeby, Imperial College London, United KingdomDipshikha Chakravortty, Indian Institute of Science (IISc), India
Copyright © 2021 Zhou and Roujeinikova. This is an open-access article distributed under the terms of the Creative Commons Attribution License (CC BY). The use, distribution or reproduction in other forums is permitted, provided the original author(s) and the copyright owner(s) are credited and that the original publication in this journal is cited, in accordance with accepted academic practice. No use, distribution or reproduction is permitted which does not comply with these terms.
*Correspondence: Anna Roujeinikova, anna.roujeinikova@monash.edu